The Water Molecule
Most of the physical properties of water that are of
interest to biologists are the result of its unique molecular
configuration. H2O is, of course, comprised of two
hydrogen atoms bonded to a single oxygen atom. Water forms in an
exothermic reaction whenever hydrogen and oxygen are mixed and
exposed to enough energy to activate the reaction. This reaction
has been known since the discovery of the two atoms, but was
demonstrated most spectacularly in an experiment carried out by
the German government. In 1933, the Germans filled a large
envelope with hydrogen, sent it across the Atlantic Ocean to
build up a large static electrical charge, and allowed the charge
to equalize with a metallic structure at Lakehurst, New Jersey.
The static discharge ignited the hydrogen in the envelope with
atmospheric oxygen to form water. The main problem with this
experiment was that it took place too close to the ground for a
really large crowd to see, and, since this was before television,
we had only radio reporters to relay the observations to
scientists around the world. However, since there was a loss of
life in the incident, some reconstructionists propose that this
elegant experiment was in fact nothing more than a terrible
tragedy. Since the German records were lost in the war, we may
never know the truth.
Once combined, the water molecule is a strange beast. The
oxygen "side" of the molecule has a slight negative
charge, while the hydrogen "sides" are slightly
positive (Fig. 1). This forms a bipolar molecule that can
bind the oxygen side loosely to a positively charged ion or
molecule, and the hydrogen side to a negatively charged ion or
molecule, thus obeying Abdul's Law that opposites attract (Abdul,
P. 1990, Chrysalis). This explains many of the unique properties
of water. It dissolves so many different things because it can
dissolve anything with an ionic charge - positive or negative. It
is so dense because under normal circumstances the adjacent
molecules are also water molecules and the oxygen on one water
molecule binds loosely to the hydrogen on the next, and the
binding pulls all the molecules together, packing them in.
Surface tension is high because of the attraction of the
molecules.
Figure 1. The water molecule, showing positive
and negative polarity
Surface Tension, Hydrophilic and Hydrophobic Interactions
Water binds to hydrophilic (water-loving)
surfaces because such surfaces have slight electrical charges; it
beads up on hydrophobic (water-hating) surfaces because
they have no charges and the water would rather mingle with
itself than mess around with something that has no charge. Oil,
for instance, is beautifully balanced ionically, and, you know,
oil and water just don't mix. Exxon demonstrated this eloquently
in 1989 with a large-scale experiment in Alaska. When some
objected that sure, it works in Alaska because it's so damn cold
up there, Exxon promptly repeated the experiment (though on a
smaller scale) at sites all over the United States. Ironically,
although oil and water don't mix, crude oil does mix with the
skin oils painstakingly groomed into the fur of sea mammals and
the feathers of sea birds, where the natural skin oil waterproofs
the keratinaceous outgrowths and allows them to trap air, which
acts as an insulator. Crude oil, however, mixes with the skin
oils and mats the hair (feathers) down flat, eliminating the air
space and the insulation. And, of course, the oil just tastes
yummy when it sits in the belly of some poor critter that tries
to lick it off so it doesn't freeze to death. Again, Exxon is
currently conducting research into these effects.
Detergents destroy surface tension by insinuating their
ions among the water molecules and binding them together more
loosely than they would normally bind. In your washer this is
good, because the detergents allow the water to get in and lift
out the soils. In a stream, this is bad, because all the water
striders suddenly sink.
Other organisms use "detergents" in creative
ways. For instance, the whirligig beetles (Gyrinidae) secrete a surfactant
- a chemical that reduces surface tension - behind them. They are
then "drawn" forward by the surface tension. Whirligig
beetles are unusual in several instances. They have 2 pairs
of compound eyes, one pair above and one pair below the water.
The also use chemicals other than surfactants. They have an
aggregation pheromone that supposedly smells like Juicy FruitTm gum (the gum can be used to
attract the beetles), and they smell like ripe apples (a
defensive secretion). They use echolocation to find each other,
avoid obstacles, and find food.
Organisms that exploit the surface tension of water are
numerous. Some use the surface tension as a platform to support
their weight above the water; others suspend themselves from the
surface of the water. For small organisms, the surface tension of
water creates a significant barrier to their movement in or out
of the water. The trick in dealing with surface tension is to
fashion biomolecules to interact with the water in an appropriate
way. Add positive and negative ions to your molecule in the right
places and you create a polar molecule which will be
hydrophilic (the water would rather bind to it than to other
water molecules); eliminate all ionic imbalances and you create a
hydrophobic molecule which will not interact with water. Vaseline
and waxes are examples of hydrophobic molecules; organisms often
use waxes to make a surface hydrophobic.
The interplay of hydrophobic and hydrophilic surfaces
with water can perhaps best be seen by examining mayflies. These
organisms have aquatic larvae with hydrophilic surfaces. These
surfaces allow the larva to exchange gasses (O2, CO2)
with the surrounding water, which can come very close to the
hydrophilic skin. At maturity, the larval mayfly molts to the
winged subimago, the stage that must break through the
water's surface. The subimago is covered with small waxy hairs
and beads that are hydrophobic. The subimago floats to the
surface and is literally pushed out of the water as the polar
water molecules attempt to reform behind the subimago. The
subimago can float comfortably on the surface of the water for
several seconds - long enough to provide a target for trout, and
the basis for fly-fishing - before taking off. It will fly to
streamside vegetation and molt again; it is the only
insect to molt once it has reached the winged stage. This second
molt gets rid of the awkward, heavy, waxy coat of the subimago.
The adult mayfly is quicker, but must avoid getting too close to
the water. Some mayflies that do come close to the water during
their later mating flights may retain some parts of the body
(particularly the underside) in a hydrofuge (hydrophobic) state.
See the paper by Edmunds and McCafferty (1988) for more details
on this interesting transition.
On the surface, water striders (Fig. 2), Collembola, and a
host of other small animals try to avoid making a
transition - that is, sinking. They all have hydrophobic bodies
with one exception - the claws. The claws are hydrophilic and
penetrate the water surface, allowing the animal to "get a
grip" on the water surface. Vogel (1988) has constructed the
"Jesus Number" - an index of the practicality of an
organism walking on water:
T
|
= surface tension |
(0.073 Newtons/meter in
freshwater,
0.078 N/m in saltwater)
|
p
|
= density of organism |
(kg m-3) |
l
|
= length (perimeter) of
organism in contact with water |
(m) |
If Je is greater than 1, it is possible for the
organism to walk on water. We won't do anything much with this
equation; other than to note that the limits on an organism are
primarily a function of its size, since surface tension, gravity
and density are all pretty much fixed.
Figure 2. A water
strider on the surface. The water displaced in the
"dimples" on the surface of the water weighs the same
as the insect. Hydrophobic hairs on the legs do not penetrate the
water surface; the surface tension holds the water together and
the water surface bends rather than breaks. An illustration of
the "Jesus number"!
Other organisms suspend themselves from the surface.
Mosquito larvae, for instance, have hydrophilic bodies with a
fringe of hydrophobic hairs near the breathing tubes at the
posterior end of the body. The hairs can be bunched into a small
tube and "punched" through the surface (or withdrawn).
On the surface, they spread out and anchor the organism to the
surface, and the larva is then able to breathe air from the
atmosphere. One method for dealing with such larvae is to cover
the surface with oil or detergents. The oil will smother the
larvae since they cannot anchor in the oil layer, which is also
hydrophobic. The detergent, of course, will lower the surface
tension (the numerator in the number Je, above).
Density and Buoyancy
Density changes in water as it warms or cools have
profound impacts on aquatic organisms. Density is also a major
component of two other important parameters - dynamic and
kinematic viscosity. All three of these parameters decrease
(usually) with increasing water temperature. Dynamic
viscosity represents the "stickiness" of water - how
easily it flows (molasses has high dynamic viscosity). Kinematic
viscosity is dynamic viscosity divided by density, a useful
parameter in some calculations because it eliminates the effects
of mass. It is easier to swim (or move blood) in warm water, but
it's harder to float.
Floating in H2O is made somewhat easier due to the
density of water. Most organisms have bodies that are at least
90% water, which means that at least 90% of their body weight
will be offset by the weight of the water around them. It's that
last 10% that is critical. Organisms that live on the bottom of a
body of water are called benthic organisms. Efficient
locomotion for them often entails walking or crawling on the
substrate. Just as on land, in order to walk on the bottom, they
need traction, and traction is largely determined by weight - or,
more accurately, the downward pull on the body caused by gravity,
which, as we have just seen, is largely offset by water for
aquatic organisms. For instance, try walking in a pool. The
shallower the water, the easier it is to walk. As depth
increases, so does the effort you must put into walking. This is
partly due to the increased effort it takes to move the water out
of your path, but you'll also notice that in deep water, over
chest height, you have a little more "bounce" in your
step, and it gets hard to walk. Benthic organisms usually deal
with such problems by making that last 10% of the body as dense
as possible; they may have heavy shells, massive bones, or even
construct cases out of rocks to carry around.
Swimming organisms (nekton), or floating
organisms (plankton), have the opposite problem, and their
solution is similar. They make the remaining 10% of their bodies
as light as possible. Fats and oils are two biological molecules
that are less dense than water and allow some organisms to float.
Fats and oils are also a storage medium for energy and can be
used for insulation in warm-blooded animals. Sharks have large,
oil-filled livers which help them float; Flipper and other
porpoises kill sharks by striking them in the liver with their
snout, rupturing the liver. Trapped air is also effective as a
float, of course. The Portuguese man-of-war has a gas float;
waterfowl float high in the water due to air trapped in their
feathers and the hollow bones in their skeletons. Fish have
air-filled swim bladders (some completely isolated from the
outside atmosphere and regulated through gas exchange with the
blood); bladderworts (a floating plant) have air filled cavities
(bladders).
Very small organisms also try to manipulate their
density, but their efforts are often more difficult to explain.
Diatoms, for instance, must stay near the surface to obtain
enough light for photosynthesis, yet they have a heavy shell of
silica. They offset the shell to some extent by a store of oil,
but they still "worry" about sinking. Fortunately, the
world of very small organisms is governed by different rules than
the world we live in. To them, water is more like molasses and
sinking rates are very small, often small enough to be offset by
local currents caused by the water heating and cooling. The
heating and cooling of the water also changes its density, and,
for small organisms in particular, this is not a trivial matter.
The change in density of water from 4o C to 25o
C is large enough to mean the difference between floating and
sinking in many organisms with densities near that of water. It
is easier to float in denser water, and fresh water is most dense
at 4o C (Fig. 3). Salt increases density of water
(Fig. 4); it is easier to float in salt water as opposed to fresh
water, and easier still in hypersaline environments such as the
Great Salt Lake in Utah. It is not unusual for populations of
small organisms to undergo seasonal morphological changes at
least partially in response to temperature induced water density
changes; one of the best examples is Daphnia (see Vogel,
1981, pages 23-24).
Figure 3. Relationship of water temperature to
density for distilled water.
Figure 4. Relationship between percent salinity
and density of water at 15o C.
The general equation governing sinking rates in water for
small spherical organisms is given below:
Where U is equal to the sinking rate, a = the
radius of the sphere, g is the gravitational constant (9.8
m s-2), p is the density of the organism, po
is the density of the medium, and m is the dynamic viscosity (kg m-1 s-1).
Note that if the density of the medium is greater than that of
the organism, U will be negative - a "floating"
rate.
Boiling and Freezing - Temperature and Heat
The fact that ice floats is due to the sudden reversal
at 4o C of the general trend of decreasing temperature
= increasing density. This is due to the ice crystals forming;
the average distance between the water molecules in ice is
greater than it is between water molecules at 4o C.
The farther the molecules are apart, the fewer will fit in any
given volume - that means less density (are you starting to
appreciate density yet?). Ice formation is discouraged by
molecules that get in the way of the water molecules - salt (Na+,
Cl-), ethylene glycol, etc. These molecules lower the
freezing temperatures of water, and allow some organisms to exist
below the "freezing point" (of pure water).
Furthermore, the freezing of water releases large amounts of
heat. This means that water is very temperature stable at the
freezing point. Winter weather is moderated to a great extent by
this factor. Winter temperatures will stay near 0o C
until all the local water is frozen. By the same token, it takes
a lot of energy to melt ice, and local temperatures will not warm
up until the ice is melted. You are probably more aware of the
other end of the spectrum - when water boils. As you well know,
the temperature of a pan in which water is boiling will not
exceed 100o C until all the water has boiled out. This
means that you can boil water in a paper cup - but watch out when
the water is gone!
Boiling is the rapid evaporation of water. Unlike
freezing, which does not occur until all the water is at 0o
C; evaporation can occur at any temperature as long as the
atmosphere is not already saturated with water. The ability of
air to hold gaseous water is also correlated to temperature; the
warmer the air, the more water it can hold. In the winter, a
house takes outside air, which is cold, and warms it. This warmer
air can hold more water, and since it is "short" of
water, water will evaporate from moist areas in the house
(plants, people, etc.) more quickly. Therefore, you have to water
plants more often, and you are more likely to catch cold, since
your respiratory linings can dry out and be unprotected from
viruses. When warm air is cooled, it can no longer hold water and
water comes out of the air. If the ground cools off, it will pick
up H2O from the adjacent air (dew); as water vapor
rises through the atmosphere it cools and the water condenses and
falls to the ground (fog, clouds, rain). Moist air also holds
more heat and "feels" warmer to us (although moist air
and dry air at the same temperature are at the same
temperature we perceive the moist air as "warmer").
The large number of molecules per unit of volume
(density) of water means that the overall kinetic energy of all
those molecules combined (heat) will also be large. In addition
to the "extra" heat that must be added or extracted
when changing the physical state of water from solid to liquid to
gas (or gas to liquid to solid); it takes a lot of energy (heat)
to change the overall kinetic energy of the densely packed water
molecules. It is important to realize the difference between heat
and temperature, temperature is a quality, heat is a quantity.
Temperature measures how fast (how much kinetic energy) a group
of molecules is moving; heat measures how many molecules have
that energy. Water, being dense, has a lot of molecules,
therefore, a lot of heat, at least compared to air. The specific
formula for heat is:
H = m
x t x sh
Where H = heat, m = mass (grams), t = temperature (degrees C),
and sh = specific heat, a constant that varies from material to
material (and also varies with temperature).
We measure heat in calories, with a calorie
being defined as the heat needed to raise one gram (one ml) of H2O
at 15o C one degree C. Thus, substituting 1 for H, m,
and t in the equation above, we can see that the sh for water at
15o C is also equal to 1. The same amount of heat will
have a different effect on other materials, or on water at
different temperatures. The calorie mentioned above is different
from the caloric values assigned to food; these are roughly 1,000
calories and are thus known as kilocalories or Calories.
You should also be aware that the calorie does not exist in the
SI; it is replaced by J kg-1 K-1, or the
number of joules it takes to raise 1 kilogram one degree Kelvin
(the SI system is the official system of science and is not
the same as the metric system, although it is closer to the
metric system than the English system is). Water has more heat
than air not only because it is more dense, but also because it
has a higher specific heat - that is, if you had a kilogram of
air and a kilogram of water, it would still take more heat to
warm the water one degree than the air.
The high specific heat of water, coupled with the
amount of heat absorbed or released in changing its physical
state, combined with the vast quantities of water on the planet,
means that water plays a major role in climate and weather. Water
is the greatest sink and conveyor of solar energy reaching the
earth. For instance, heat carried by the Gulf Stream from the
warm tropics keeps England and parts of Europe much warmer than
they would otherwise be given their latitude. Organisms living in
oceans may only encounter a 2 - 3o C temperature
change over their lifetimes. Organisms living in sizable
(pond-size) bodies of water do not experience diurnal temperature
changes (except at the surface and edges), and only a 30o
C change seasonally, spread out over a period of weeks.
Terrestrial organisms may experience a 30o C
temperature change daily - especially in deserts where there is
little water vapor in the air to block heat transfer - more on
that later.
Water and Light
Water interacts with the light that strikes it. These
interactions are very complex, and extremely variable, and we
will only briefly discuss them here. Pure water tends to absorb
long wavelength light (reds, infrared) strongly, and allows short
wavelength light to pass (blues, ultraviolet)(Figure 5, below). Any suspended or
dissolved particles in the water increase absorption,
particularly of UV (ultraviolet) light. Since the concentration
of such suspended or dissolve materials varies greatly, few
general principles exist. However, it is generally accepted that
at depth, particularly in the ocean, the light has a bluish cast
and underwater photography relies heavily on flash or artificial
light to image the reds and oranges that otherwise appear black
at depth due to the lack of those colors in the ambient light.
Fish and other organisms that appear brightly colored to us when
brought to the surface may actually be quite drab in their native
habitat. This factor cannot be overemphasized when exploring the
biological significance of color in aquatic organisms - they must
not be judged in terms of their surface coloration (also, unless
you know something about the color perception of the organisms in
question, coloration is meaningless).
Water color itself is also a tricky subject. Pure water
appears clear. Its surface may take on the appearance of the sky
through reflection, or of the substrate underneath due to
refraction, and the two - reflection and refraction - interact as
the viewer changes angles relative to the water surface. Water
may also take on the color of materials suspended in it,
including algae and sediment. The lovely color of the Ohio River
is not a reflection of the sky (that would make it gray), or even
a refraction of the bottom (which you can't see even in water
centimeters deep).
It should come as no surprise that water transmits the
same colors - blues - as are vitally critical in photosynthesis;
after all, photosynthesis evolved in water. Our traditional view
of the two-peaked photosynthetic absorption curve, with peaks in
the red and blue, is somewhat distorted by the presence of
accessory pigments that increase absorption of long-wavelength
light. Also, remember that light passing through natural water is
also being absorbed by photosynthetic organisms, which means that
there is competition for light at greater depths by
photosynthetic organisms.
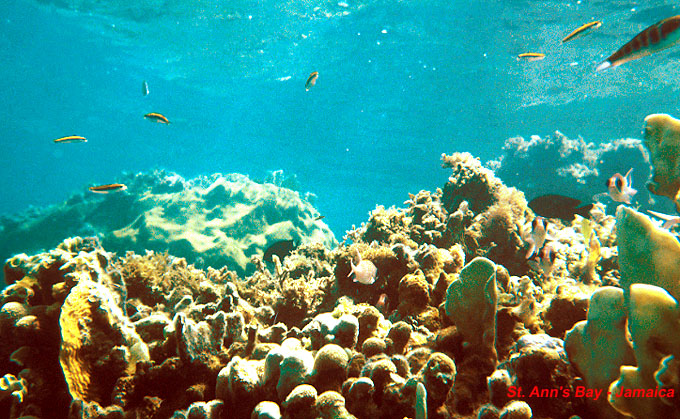 |
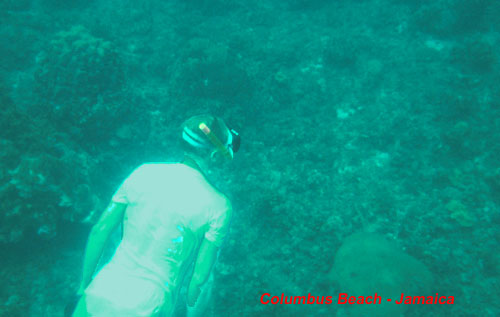 |
Figure 5. Colors
and water. The photo to the left was taken near the surface. With
little water to penetrate, reds and yellows have not been absorbed and you
can see yellows among the corals in the foreground. Objects in the
distance appear bluish because yellow and red light was absorbed between
those objects and the camera. In the photo to the right, the
snorkeler's white t-shirt takes on a bluish cast as the red and yellow
light needed to constitute white light have been absorbed. The
actual depth is about 10 feet (3 meters). |
All in all, however, the color of light in water is not
as critical to photosynthetic organisms as the intensity of that
light. Near the surface there may actually be too much light; at
depth there may not be enough. Of crucial interest to us will be
the phytoplankton, those organisms such as algae that
photosynthesize, yet are at the whim of the currents as they lack
the ability to swim strongly. The depth at which a photosynthetic
organism's rate of photosynthesis balances its rate of
respiration is known as the light compensation point
(LCP); a small organism with little stored energy reserves cannot
survive long if it sinks below its LCP. Note that the LCP will
vary by species and that dissolved or suspended materials
(including other phytoplankton) in the water column will absorb
light and raise the LCP for all species - the LCP in the
Caribbean is measured in tens of meters; in the Ohio River it is
measured in centimeters. The cyanobacterium Plectonema woolei
in turbid Lake Erie has virtually all the known accessory
pigments. Grown under low-light conditions (it can grow in a
refrigerator, apparently getting enough light when the door is
opened occasionally) it is black in color. Its LCP in Lake Erie
is often about 10 m, where visibility to the human eye is
measured in cm.
Modification of the LCP of many organisms will, of
course, have profound impacts on the ecosystem. One of the more
sinister projections of a "nuclear winter" scenario is
the decreased light penetration due to smoke and dust in the
atmosphere. This would raise the LCP to near (above?) the surface
of the ocean, and such effects would persist for a longer period
of time than the small phytoplankters, with their tiny energy
reserves, could hold out. They would die, and since they produce
a major portion of the O2 in our atmosphere .....
Figure 6. Light extinction vs. depth.
The lines above trace the relative light intensity from the surface to a depth
of 25 meters (the spreadsheet calculated the intensity every 1/10 meter down to
10 meters then every meter after that). The clearer the water (open ocean,
Lake Tahoe) the less light is absorbed. Very eutrophic (enriched) habitats
with a lot of plankton in the water (James River Estuary, Lake Erie in 1974)
have a lot of light absorbed. Compare the line for Lake Erie in 1974 and
1995 (when it was the 3rd cleanest among these water bodies). The
difference? Zebra Mussels filtering out the phytoplankton (as well as a
lot of money put into sewage treatment in the US and Canada),
As light passes through water in the air, similar
effects are seen. Water vapor in the air absorbs infrared (IR)
strongly, while allowing UV to pass; thus, a cloud blocking the
sun causes us to perceive a drop in temperature, and it is
possible to get sunburned on a cloudy day. Water vapor also
absorbs heat trying to leave the Earth and radiate out into
space. One reason that deserts are cold at night is that there is
little water vapor to block heat loss at night (the same heat
loss occurs during the day, but is offset by incoming solar
radiation, insolation); for the same reason, it gets
cooler on clear nights than on cloudy ones. Water plays still
another role in the global heat balance. Light colored objects
reflect light (and heat); thus clouds, ice and snow, which are
light-colored (have a high albedo), reflect heat back into
space and tend to cool the planet. If you have a lot of snow, as
during an ice age, this effect can feed on itself: the snow
reflects the heat, which cools the planet, which allows more snow
to form, which further cools the planet, and so on. The reverse
takes place if things warm up, an important consideration in our
examination of global warming.
Pressure
We have not mentioned pressure yet, primarily because
it is of less concern to aquatic organisms than you might think.
Although there are real physical/chemical effects that occur at
great pressures, the biggest problems that organisms face is a difference
in pressure, and most organisms deal with this very effectively.
Pressure is caused by the weight of fluids (air, water), above
you. At sea level, we measure pressure as one atmosphere (we'll
avoid the messy SI units, pascals m-2, for now). That
is the cumulative pressure of all the air. By the time we
go only 10 m underwater, the pressure doubles to 2 atmospheres,
and it increases by 1 atmosphere every additional 10 meters.
While water is (nearly) incompressible, air is not, and air at
atmospheric pressure taken underwater soon begins to compress;
here is where most pressure-related problems come into play. An
organism using air for buoyancy will find that air compressed as
it sinks, further decreasing buoyancy and thus increasing the
sinking rate, leading to a positive feedback loop. Any organs
(lungs) containing air will be crushed as the air inside can no
longer support them. Rising through the water column can have the
opposite effect: uncontrollable ascent rates and explosion of air
containing tissues. Organisms with air-containing organs are well
advised to limit their vertical movement in the water column, or
to enclose their air in a hard structure, such as the shell of a Nautilus.
Fish caught in deep-sea trawls often explode as their swim
bladders expand when they are forcibly brought to the surface.
Whales, such as the sperm whale, and other organisms that
routinely dive to great depths and return to the surface to
breathe, have other adaptations that prevent damage (such as the
lungs being small relative to the overall mass of the whale).
Humans entering water without any special equipment are
limited to relatively shallow depth (30 m) for short periods of
time (60 s). This was changed by the introduction of compressed
air. Compressed air has enough pressure of its own to compensate
for the pressure of the water, and allows the lungs to expand
normally. As long as the compressed air is the same pressure as
that of the surrounding water, and as long as the air can move
through all air passages in the body freely (there is no
congestion in the sinuses or eustachian tubes), all is well - up
to a point. If the diver ascends without breathing normally, the
air in the lungs is at a greater pressure than the surrounding
water, and the lungs expand to the point that the thin tissues of
the aleovi rupture, leading to air in the blood (and blood in the
lungs), and probable death by air embolism. This effect is
particularly pronounced in shallow waters, where a change in
depth of a few feet without normal breathing can cause an
embolism, often without accompanying pain, thus making it very
dangerous. Most SCUBA (self-contained underwater
breathing apparatus) classes simply involve ramming home
the simple rule of breathing normally at all times, and allow the
diver to become proficient enough that panic is not likely to
occur and cause the diver to forget that simple rule.
From a physical standpoint, a human with compressed air
could go to the deepest points of the ocean. From a physiological
standpoint, however, this is not practical for several reasons.
One is that oxygen is toxic, and the critical factor is the
partial pressure of O2. Without going into details,
this means that as depth increases, O2 becomes more
toxic. To avoid this, divers going to depths below 100 m
routinely use gases with less than "normal" 21% oxygen.
Nitrogen, normally inert in the body, causes neurological effects
(Nitrogen narcosis) at depths greater than 100 m, and is often
replaced by helium (which carries body heat away so effectively
that special provisions must be made to warm the diver - He also
causes "Daffy Duck voice). Helium-oxygen mixtures have been
tested to depths of about 600 m. Because of the costs of the
specialized equipment and support teams, as well as the problem
of decompression (see below), 600 m may be a practical limit for
humans in the ocean. Below that depth humans need to be encased
in structures (submarines, hard diving suits) that bear the
pressure of the water, leaving the human at normal pressure.
Recent advances in electronics have also made remotely piloted
vehicles (ROV's) practical for work at great depths; witness the
recent success of the ROV Jason in the discovery of the Titanic.
A further complication of diving with compressed gases
is that the gasses form tiny bubbles in the blood under great
pressure. As the diver ascends, these bubbles come out of
solution and grow rapidly, not unlike the way a carbonated
beverage bubbles when you remove the top (ever play beer
hunter?). Like such beverages, agitation increases bubble
formation, and the bubbles are most likely to form at the joints.
The bubbles block blood flow and pinch nerves, causing the victim
great pain, particularly at the joints. Victims often assume a
bent, fetal position, giving rise to the common name for this
syndrome - the bends. It often afflicted workers in caissons,
pressurized structures built on the bottom of a body of water to
facilitate construction work (bridge pylons, tunnels). A
celebration of the completion of one such structure (a tunnel
under the river in New York) turned out rather flat when the
politicians found that the champagne wouldn't fizz under
pressure. Fortunately, the local populace was treated to a joyous
moment sometime later, when the politicians emerged and their
otherwise boring speeches were punctuated by enormous belches
fueled by great quantities of now depressurized champagne.
Treatment for the bends requires repressurization in a
recompression chamber, a fact known to all fans of Sea Hunt,
Flipper, or Voyage to the Bottom of the Sea.
Avoiding the bends requires carefully planned decompression stops
on ascent, allowing the gasses to be vented slowly, a fact also
well known to most fans of the above shows. Lesser known is that
most sport divers are counseled to avoid decompression by
planning no-decompression dives; these carefully limit the times
spent at different depths to avoid build-up of N2 in
the blood. A no compression dive might mean being able to stay
down 1 hour at 10 meters, but only 15 minutes at 20 meters. Time
spent ascending, descending, on the surface, and flying on
airplanes must also be considered. The charts formerly used to
calculate no-decompression (and decompression) dives are being
replaced by microcomputers worn by the diver which sense depth,
altitude, and time, and advise the diver accordingly. Lesser
known still is that the research on which these charts are based
was performed using US Navy divers during and after WWII. These men
were usually about 2 m tall and had virtually no body fat,
consequences of stringent recruiting practices and physical
training. Recent research has shown that factors such as sex,
size, and body fat can influence onset of the bends. Body fat, in
particular, has an aggravating effect on the bends, and the
relative safety of sport diving up until this time has been due
to the extreme conservatism of the Navy scientists (who also
fudged the data so that the dumber of these big guys could still
use the tables in an era when an electronic calculator filled a
room). The gender bias of the study was understandable given who
was developing these tables, and what their motivation was; what
is inexcusable was the length of time it took to replicate these
studies with women (and people of different sizes and fat
contents). Such data is being gathered and incorporated into such
tables now.
Drag
A final physical topic related to water is the cost of
moving through it. We have already seen that vertical movement -
buoyancy or sinking - is easily accomplished by manipulating
density and letting the forces of gravity do the rest. Horizontal
movement, or vertical movement against the forces of gravity, is
more complex. Two things must happen when an object moves through
water. The water in its way must be moved, and the water
"sticking" to it must be sloughed off. The first of
these is a function of speed, size, and shape of the organism and
is called pressure drag; the second is a function of speed
and the amount of surface area present and is called friction
drag; together they are called simply drag. As speed
increases, or as size of the organism increases, friction drag
becomes less important than pressure drag. This is due to the
fact that friction drag is proportional to the surface area of
the organism, while the amount of water that must be moved is
more proportional to the volume, and, as size increases, volume
increases more than surface area. Friction drag is also more
important in air than in water, and at slow speeds in relation to
high speeds. The type of drag that predominates can have dramatic
consequences for the organism. For instance, we live in a world
dominated by pressure drag, and the way to reduce pressure drag
(thus increasing efficiency of locomotion) is to streamline
an object, making it present a small profile to the fluid the
object is moving in - a Corvette has less pressure drag than a
Mack truck, even if both were the same size. However,
streamlining also increases friction drag. In our world of big
things and high speeds, friction drag is by far the smaller
coefficient of overall drag and can be ignored, but many small
organisms live under the reverse conditions and cannot afford to
overlook friction drag.
How can we tell which type of drag will be most
important to an organism? Fortunately, a simple mathematical
relationship exists, the Reynolds number (Re):

Where p = density of the fluid; l =
characteristic length of the object; U = the speed (of the
object or the fluid or both); and m =
the dynamic viscosity. The length is somewhat arbitrary; it is
usually the length normal to the flow, i.e. the diameter of a
sphere, the diameter of a cylinder normal to the flow, the length
of a cylinder parallel to the flow. It is important to set up the
equation so that all of the variables are in the same units -
that is, you can't have a speed measured in meters per second and
a length measured in millimeters. Under these conditions, Re is a
dimensionless index (try it, all the dimensions cancel out). If
Re<1, then friction drag predominates and flow is smooth or laminar.
Between 1 and 1000, there is a transitional zone, where both
factors are important but hard to predict (unfortunately, most
organisms spend at least part of their lives in this zone), and
flow may become turbulent (turbulence is a major factor in
pressure drag). Above 1,000, pressure drag predominates, and you
would expect to see streamlined structures (which work, in part,
by avoiding turbulence which predominates at high Re).
Note the effect of changing any variable. Water is more
dense than air, it leads to a bigger Re (the effect of the
dynamic viscosity is swamped by the larger values for density);
longer organisms will have a larger Re; larger Re's will
predominate at higher speeds. Life at low Re is a strange world;
recent experiments to simulate low Re conditions for humans used
lime Jell-O (high viscosity) and have been perverted into a
strange ritual staged as "entertainment" where
opponents attempt to find optimum movement strategies for the low
Re and best their opponents in physical contests. Apparently,
wearing a minimum of clothing has been found to be adaptive under
such conditions, perhaps clothing increases friction.
The Reynolds number has far-reaching effects, and we
will discuss many of them this semester. One we have already
discussed is sinking rates:
Once the sinking rate is calculated, it is necessary to use
the value of U to calculate a Re for the spherical organism. If
the Re > 1, the calculated sinking rate may not be accurate,
since the equation for sinking rate assumes the laminar
conditions of low Re. If the Re is greater than 1, turbulence may
develop, increasing pressure drag and reducing the sinking rate.
There are formulas for the sinking rate of larger (or faster
sinking) objects, but we won't go into them here.
Further Reading - Physics
McShaffrey, D. and W.P. McCafferty. 1987. The behavior and form of Psephenus herricki (DeKay) (Coleoptera:
Psephenidae) in relation to water flow. Freshwater Biology. 18:319-324.
Craig,
Douglas A. Some of what you should know about water or,
K.I.S.S.* for HYDRODYNAMICS (*Keeping It
Stupidly Simple)
Department of Entomology, University of Alberta, Edmonton, CANADA T6G 2E3
- Cole, G.A. 1983. Textbook of Limnology. 3rd. Ed.
Waveland Press, Prospect Hts., IL, 401pp.Read Chapters
8-9
- Edmunds, G.F. and W.P. McCafferty. 1988. The mayfly
subimago. Annual Review of Entomology 33:509-529.
- Gleick J. 1987. Chaos: making a new science.
Viking Penguin Inc. New York. 352 pp.
- McCafferty, W.P. 1981. Aquatic Entomology Science
Books Intl., Boston. 448 pp.
- Vogel, S. 1981. Life in Moving Fluids Princeton
University Press, Princeton. 352 pp.
- Vogel, S. 1988. Life's Devices Princeton
University Press, Princeton. 367 pp.
|